Love! What is love
if not knotted in garlic? Child, we move through graves
like eels, delicious with our heads first, our mouths
agape.1
–Jane Wong
In the age of modernity, and in a nation with a sizable food surplus, how many of us can claim to have enjoyed the consistent feeling of satiety, or fullness, for more than a few generations into the past?2 Whether the cause is ammonia production, which feeds roughly 40% of the global population through one extremely viable fertilizer, or the Green Revolution, represented by a huge uptick in high-yield grain crops, the human diet has clearly shifted.3, 4 Health staples such as fruits and vegetables overflow from grocery store shelves, and unlike our prehistoric ancestors, many of us don’t need to wait for an abundant season to have our fill of them. And yet, metabolic diseases related to obesity and poor nutrition are commonplace in the United States.5, 6 As people eat more, for better and worse, important metabolic questions arise regarding the effect of our changing diets on human biological machinery. Famines are not infrequent occurrences, and they often leave in their wake a visceral memory of hunger.7 What does a legacy of starvation do to modern, food-rich generations, especially when the mechanisms of the body are designed for consumption? It is this question of “metabolic programming” that many researchers seek to understand, as studies suggest that our bodies can fight stressors like famine across generations, even after they have long since disappeared.8
Intergenerational vs. Transgenerational
The first key terms to distinguish here are intergenerational and transgenerational effects, both of which reference different conditions in metabolic studies. Intergenerational effects are those conveyed in response to stress exposure through the germline, the direct parental generation. Transgenerational effects are conveyed in response to triggers not directly present in the parental generation or the environment; rather, these effects are inherited stress responses, written into ancestral metabolic chemistry. As indicated in Figure 1, the phenotype of yellow fur, for example, is represented differently between modes of inheritance in offspring, depending on their distance from the original trigger.9
Evidence of the intergenerational inheritance of stress responses, specifically the metabolic response to nutrient deprivation, is well-observed in retrospective studies.8 Tragedies such as the “Dutch Hunger Winter” offer clear insights into the cause-and-effect relationship between parental stress and physiological illness in offspring. In a 1976 study, the children of pregnant women who experienced the Dutch famine (1944-1945) under German occupation were traced 50 years into the future, into a period of postwar prosperity. Fetal malnutrition was already known to lower birth weight, infant length, head circumference, and other important indicators of health. However, in a key discovery, scientists observed an association between “exposure to famine during any period of gestation” and “reduced glucose tolerance and an increased risk of type 2 diabetes.”10 More recent studies have clarified this finding, suggesting that metabolic pathways are especially sensitive to maternal malnutrition during peak periods of cell division, specifically during the development of the endocrine pancreas in the womb, whose β cells secrete the glucose regulator insulin.10, 11 Descendants of famine can experience can thereby experience metabolic abnormalities indicative of a stress response, such as increased risk of Type 2 Diabetes—despite their lack of direct exposure to famine. These individuals are often cited as an early, poignant discovery in the field of human development.12 While there is varying evidence of transgenerational effects in this case, it is this discovery that opens the door to more focused research on the generational impact of food deprivation.13
With regard to transgenerational effects, the difficulty often lies in separating cultural and social factors from that of metabolic or epigenetic factors.9 Starvation can, and often does, have huge interpersonal ramifications that can be mistaken for casual links to nutrient deprivation. To mitigate this cultural influence, and to demonstrate the mechanistic pathways of metabolic programming, animal models provide excellent “proof-of-concept” insights into the effects of starvation on future generations.8
Metabolic Changes in C. elegans
The animal Caenorhabditis elegans (C. elegans) is a roundworm particularly well-suited for generational studies of metabolic programming. A 2023 paper revealed a clear mechanistic basis attributed to increases in metabolic abnormalities observed in the offspring of starved C. elegans. This discovery illuminated specific molecular pathways responsible for changes in phenotypes. The study used what was referred to as a “starvation paradigm,” in which L1-stage animals, or early juvenile larvae, were hatched in the absence of food for six days—a practice that arrests their development—before being fed and allowed to develop normally. To maximize exposure of early-life starvation, this was repeated for five consecutive generations, labelled as P0L1starved (the parental generation) to F4L1starved (the fifth generation). The next two generations were well-fed (F4L1starved + 1fed and F4L1starved + 2fed), and the following generation (F4L1starved + 3fed) was analyzed in separate groups under well-fed or starvation conditions (Figure 2).8
One of the mechanisms studied in these generationally-starved roundworms was insulin/IGF signaling (IIS) activity, which is responsible for key metabolic pathways related to aging, development, behavior, and, importantly, fat accumulation.8, 14 Proteins that bind to specific DNA sequences, thereby regulating gene expression, are referred to as transcription factors. The main transcription factor that results from IIS is DAF-16/FOXO. Acute starvation in L1 is shown to increase the presence of DAF-16/FOXO in somatic tissues, heightening DAF-16/FOXO activity through adulthood. This results in a decreased resistance to oxidative stress, leading to physiological changes in future generations (Figure 2). Notably, if the parental generation had depleted levels of neuronal DAF-16/FOXO, the F4L1starved + 1fed and F4L1starved + 3fed animals had improved oxidative stress resistance.8 Oxidative stress plays a role in a wide range of diseases, from atherosclerosis and Alzheimer disease to cancer, and this transgenerational effect on stress resistance is critical to understanding the impact of metabolic programming on disease susceptibility.15
The Complexity of Human Disease
Questions related to the transgenerational effect of obesity among food-rich generations, especially those that rely on retrospective data models, provide clear guidelines for the future of metabolic research. Studies have already noted a sex-linked basis for starvation-induced metabolic programming in humans, related both to the sex of the parents exposed to the stressor and the sex of the offspring in the following generations (Figure 3).7 In addition, there is evidence across various animal models of similar “metabolic, cardiovascular, and even neurological impairments” in the offspring of undernourished and overnourished mothers, suggesting that metabolic programming follows predictable and conserved pathways.8 However, the complexity of inheritance in these studies is not fully understood on a molecular level. In order to clarify the specific epigenetic connection between our metabolic past and present, more research needs to be done on the mechanisms responsible for these changes. Animal models, like C. elegans, are excellent for this research and have already provided incredible insights into known disease patterns in humans. Considering the impact these findings can have on our health and longevity, it is critical that we continue to explore the role of our ancestors in our modern relationship with food and take steps to mitigate their effect on future generations.
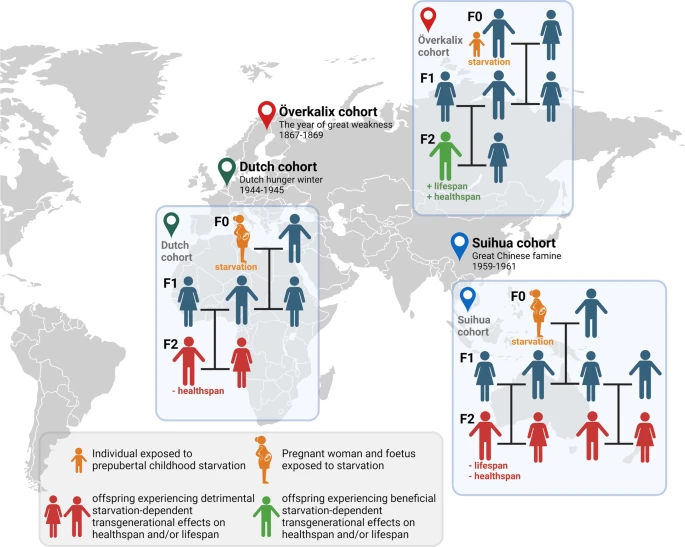
Acknowledgments
I would like to thank Catherin DeSousa, a Metabolic Biology PhD student at UC Berkeley and Graduate Student Instructor, for kindly taking the time to review this article and provide interesting and meaningful feedback.
References
- Wong, J. (2019). After Preparing the Altar, the Ghosts Feast Feverishly. The Poetry Foundation. https://www.poetryfoundation.org/poetrymagazine/poems/148098/after-preparing-the-altar-the-ghosts-feast-feverishly.
- Huang, C.-H., Liu, S.-M., & Hsu, N.-Y. (2020). Understanding Global Food Surplus and Food Waste to Tackle Economic and Environmental Sustainability. Sustainability, 12 (7), 2892. https://doi.org/10.3390/su12072892.
- Patil, B. S., Hessel, V., Lang, J., & Wang, Q. (2016). Plasma-assisted nitrogen fixation reactions. Alternative Energy Sources for Green Chemistry, 296–338. https://doi.org/10.1039/9781782623632-00296
- Ritchie, H., & Roser, M. (2024). Yields vs. Land Use: How the Green Revolution Enabled Us to Feed a Growing Population. Our World in Data.
- World Health Organization. (2003). Diet, nutrition and the prevention of chronic diseases (Tech. Rep. No. 916). World Health Organization.
- Brown, A. G. M., Esposito, L. E., Fisher, R. A., Nicastro, H. L., Tabor, D. C., & Walker, J. R. (2019). Food Insecurity and Obesity: Research Gaps, Opportunities, and Challenges. Translational Behavioral Medicine, 9 (5), 980–987. https://doi.org/10.1093/tbm/ibz117.
- González-Rodríguez, P., Füllgrabe, J., & Joseph, B. (2023). The hunger strikes back: An epigenetic memory for autophagy. Cell Death & Differentiation, 30(6), 1404–1415. https://doi.org/10.1038/s41418-023-01159-4
- Vogt, M. C., & Hobert, O. (2023). Starvation-induced changes in somatic insulin/IGF-1R signaling drive metabolic programming across generations. Science Advances, 9(14), eade1817. https://doi.org/10.1126/sciadv.ade1817
- Fitz-James, M. H., & Cavalli, G. (2022). Molecular mechanisms of transgenerational epigenetic inheritance. Nature Reviews Genetics, 23(6), 325–341. https://doi.org/10.1038/s41576-021-00438-5
- De Rooij, S. R., Bleker, L. S., Painter, R. C., Ravelli, A. C., & Roseboom, T. J. (2022). Lessons learned from 25 years of research into long-term consequences of prenatal exposure to the Dutch famine 1944–45: The Dutch Famine Birth Cohort. International Journal of Environmental Health Research, 32(7), 1432–1446. https://doi.org/10.1080/09603123.2021.1888894
- De Rooij, S. R., Painter, R. C., Phillips, D. I. W., Osmond, C., Michels, R. P. J., Godsland, I. F., Bossuyt, P. M. M., Bleker, O. P., & Roseboom, T. J. (2006). Impaired insulin secretion after prenatal exposure to the Dutch famine. Diabetes Care, 29(8), 1897–1901. https://doi.org/10.2337/dc06-0460
- Schulz, L. C. (2010). The Dutch Hunger Winter and the developmental origins of health and disease. Proceedings of the National Academy of Sciences, 107(39), 16757–16758. https://doi.org/10.1073/pnas.1012911107
- Painter, R., Osmond, C., Gluckman, P., Hanson, M., Phillips, D., & Roseboom, T. (2008). Transgenerational effects of prenatal exposure to the Dutch famine on neonatal adiposity and health in later life. BJOG: An International Journal of Obstetrics & Gynaecology, 115(10), 1243–1249. https://doi.org/10.1111/j.1471-0528.2008.01822.x
- Biglou, S. G., Bendena, W. G., & Chin-Sang, I. (2021). An overview of the insulin signaling pathway in model organisms Drosophila melanogaster and Caenorhabditis elegans. Peptides, 145, 170640. https://doi.org/10.1016/j.peptides.2021.170640
- Forman, H. J., & Zhang, H. (2021). Targeting oxidative stress in disease: Promise and limitations of antioxidant therapy. Nature Reviews Drug Discovery, 20(9), 689–709. https://doi.org/10.1038/s41573-021-00233-1
Image References
- Banner Image: Unsplash. (2017, March 17). Photo by Avel Chuklanov on Unsplash. https://unsplash.com/photos/photo-of-people-eating-rice-sedJnjrUMM8
- Fitz-James, M. H., & Cavalli, G. (2022). Molecular mechanisms of transgenerational epigenetic inheritance. Nature Reviews Genetics, 23(6), 325–341. https://doi.org/10.1038/s41576-021-00438-5
- Vogt, M. C., & Hobert, O. (2023). Starvation-induced changes in somatic insulin/IGF-1R signaling drive metabolic programming across generations. Science Advances, 9(14), eade1817. https://doi.org/10.1126/sciadv.ade1817
- González-Rodríguez, P., Füllgrabe, J., & Joseph, B. (2023). The hunger strikes back: An epigenetic memory for autophagy. Cell Death & Differentiation, 30(6), 1404–1415. https://doi.org/10.1038/s41418-023-01159-4