Regenerative medicine is one of the most recent and emerging branches of medicine centered around replacing diseased or damaged tissue via innovative technological and biological techniques. A promising pathway in regenerative medicine focuses on harnessing the limitless potential of human stem cells—cells capable of transforming into any of the body’s various cell types, from cardiac muscle cells to neural cells. By understanding the fundamental mechanisms of stem cell versatility, developing cellular therapies to treat currently incurable diseases is on the horizon. Specifically in the realm of neuroscience, the potential of stem cells for treating patients with neurodegenerative disorders such as Alzheimer’s has led to novel research in the field. By uncovering and understanding the intrinsic mechanisms of how stem cells function, it may soon be possible for one to artificially stimulate the creation of neurons and rebuild the human brain.
How do Stem Cells Work?
Stem cells are unspecialized cells with the power to differentiate, or transform, into over 200 different specialized cell types as required by the body. Based on their differentiation capabilities, stem cells are classified as either totipotent, pluripotent, multipotent, or unipotent. Pluripotent stem cells are given the most attention with their unique potential to transform into almost any cell type by serving as the foundational unit for the three primary germ layers that form during human development: the ectoderm (which gives rise to the nervous system and epidermis), the endoderm (which forms the gut and many of the internal organs), and the mesoderm (which becomes the muscles cells and connective tissue of the body).1

Although deriving stem cells initially required the use of an embryo, the breakthrough advancements made by Shinya Yamanaka in 2006 have allowed for the reprogramming of adult somatic cells back into their undifferentiated, pluripotent state. By taking an adult cell and culturing it with yamanaka factors—“reprogramming” factors—the cell becomes an induced pluripotent stem cell (iPSC), and may be re-differentiated into a specific cell type. Specifically, these yamanaka factors include the Oct3/4, Sox2, Klf4, c-Myc proteins, and the overexpression of these factors in a mouse or human somatic cell may induce its reversion to a pluripotent state.2
Research demonstrates that the targeted differentiation of stem cells is made possible by artificially mimicking the embryonic environment to induce a particular transformation. In one study, for example, neural stem cells were generated from mouse pluripotent stem cells. Expressing neuroectoderm-specific protein factors and signaling molecules, such as Sox1 and Six3, in pluripotent stem cells facilitated their differentiation into neural stem cells, thus creating the possibility of forming new neurons.3 This technology involving directed differentiation has a myriad of implications for the development of future regenerative medicinal treatments, specifically for patients with neurodegenerative diseases. By streamlining neurogenesis — the process of creating new neurons from neural stem cells — one may effectively replace the damaged or diseased neural cells involved with various neurodegenerative disorders, such as Alzheimer’s disease (AD). However, much more remains to be discovered with respect to the various complex molecular mechanisms that govern neurogenesis before therapeutic treatments may be made available for patients.
Although deriving stem cells initially required the use of an embryo, the breakthrough advancements made by Shinya Yamanaka in 2006 have allowed for the reprogramming of adult somatic cells back into their undifferentiated, pluripotent state. By taking an adult cell and culturing it with yamanaka factors—“reprogramming” factors—the cell becomes an induced pluripotent stem cell (iPSC), and may be re-differentiated into a specific cell type. Specifically, these yamanaka factors include the Oct3/4, Sox2, Klf4, c-Myc proteins, and the overexpression of these factors in a mouse or human somatic cell may induce its reversion to a pluripotent state.2
Research demonstrates that the targeted differentiation of stem cells is made possible by artificially mimicking the embryonic environment to induce a particular transformation. In one study, for example, neural stem cells were generated from mouse pluripotent stem cells. Expressing neuroectoderm-specific protein factors and signaling molecules, such as Sox1 and Six3, in pluripotent stem cells facilitated their differentiation into neural stem cells, thus creating the possibility of forming new neurons.3 This technology involving directed differentiation has a myriad of implications for the development of future regenerative medicinal treatments, specifically for patients with neurodegenerative diseases. By streamlining neurogenesis — the process of creating new neurons from neural stem cells — one may effectively replace the damaged or diseased neural cells involved with various neurodegenerative disorders, such as Alzheimer’s disease (AD). However, much more remains to be discovered with respect to the various complex molecular mechanisms that govern neurogenesis before therapeutic treatments may be made available for patients.
What exactly is Neurogenesis?
Neurogenesis is the incredible process by which new neurons are formed from the differentiation of neural stem cells (NSCs). It is important to note, however, that NSCs may follow two distinct differentiation pathways which dictate what type of cell differentiation will occur. Through neurogenesis, neurons (the main functional unit of communication across the brain) may be produced, and through gliogenesis, astrocytes (cells which provide synaptic support and hold nerve cells in place) and oligodendrocytes (cells which allow for the rapid conduction of nerve impulses across the brain’s neural network) may be made.4 While most important during embryonic development, neurogenesis is a process that continues throughout adulthood and is postulated to maintain healthy brain function and aid in the regulation of mood, memory, and learning.5 The process occurs primarily in the lateral subventricular zone (the largest neurogenic zone in the brain where neuroblasts, or premature neurons, are made) and the subgranular zone of the hippocampus (the region involved with learning and memory). In order to artificially stimulate this process and promote neuronal building, it is necessary to learn the fundamentals of the robust nature of adult neurogenesis. By exploring the neurobiology of how the body creates new neural cells, there exists a significant potential for the development of cellular treatments for neurodegenerative diseases such as Alzheimer’s, Parkinson’s, or Huntington’s Disease, all of which may be due in part to a fault in the neurogenic process.6


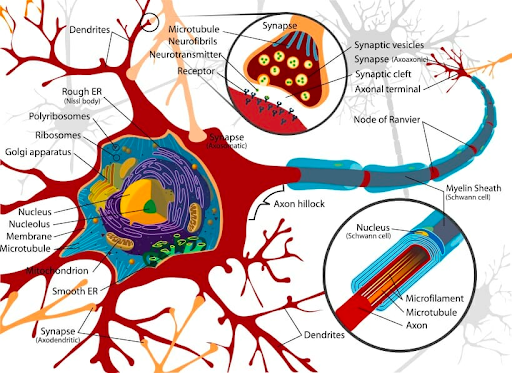
How does Neurogenesis Work?
While the full scope of the complex neurobiological processes involved in neurogenesis are still being studied, much headway has been made in understanding the integral proteins and molecules associated with the development of neural cells.
One such class of proteins found to be crucial to neurogenesis regulation is the Cyclin D family of proteins. Cyclin D proteins are regulatory proteins which control cell cycle progression and specific cyclin-dependent kinases, molecules which are necessary for enzymatic activity and the growth of new cells. In an experiment involving mice, the cyclin D2 protein was found to play a pivotal role in neurogenesis. By comparing wild-type (normal) mice to mutated mice (which had the cyclin D2 protein knocked out), it was discovered that the resulting brain structures varied significantly in size by around 25%. Brain regions such as the hippocampus, occipital cortex, and cerebellum were all found to be much smaller in weight, as compared to the normal brain. Although the molecular and chemical mechanisms are not as clear, the results of this study indicate that the lack of functional cyclin D2 protein in the adult brain ultimately results in a near-complete absence of proliferation of neuronal precursors. Thus, this protein is an important component in the process of adult neurogenesis and may inhibit the development of new neurons if impaired or absent.8

Cell signaling, or the transfer of information from one cell to another via proteins and molecules, has also been found to play a crucial role in neurogenesis. Specifically in the subgranular zone of the hippocampus (SGZ), juxtacrine, cell-to-cell contact signaling of the protein ephrin-B2—which is responsible for neuronal development—via astrocytes was found to positively regulate hippocampal neurogenesis. NSCs in the hippocampus were found to be rich in EphB4, the receptor to the ephrin-B2 molecule. At sites of cell-cell contact between NSCs and astrocytes, the ephrin-B2 released from astrocytes mediates a proneuronal effect, ultimately leading to neurogenesis. In experiments with mice, it was shown that ephrin-B signaling not only elevates adult hippocampal neurogenesis, but also plays a role in instructing NSC neuronal differentiation, or the commitment to a neuronal fate in the NSC lineage.9 Other cell signaling molecules called neurotrophic growth factors have also been found to play a major role in stimulating the differentiation of neural stem cells. Factors such as the nerve growth factor (NGF), brain derived neurotrophic factor (BDNF), glia-derived nerve factor (GDNF), and insulin-like growth factor 1 (IGF-1), all play a role in adult neurogenesis through the ultimate regulation of fate specification of neural stem cells. Neurotrophic growth factors work by activating tropomyosin-related kinase (Trk) receptors, which stimulate a cascade of chemical signaling pathways that results in the proliferation and differentiation of NSCs to create new neurons.10, 11 In common neuropathologies such as AD, where impaired neurogenesis is a common clinical precursor, these neurotrophic factors were reported in reduced levels in addition to Trk signaling. Enhancing the presence or effectiveness of these various components to increase cell signaling efficiency may thus provide many therapeutic benefits in enhancing neurogenesis, and future research may shed more light on how to effectively control and scale these reactions to develop promising treatments for patients with neurodegenerative disorders . 12
The Future of Neurogenesis in Regenerative Medicine
The process of neurogenesis is an incredible phenomenon with vast potential in the field of regenerative medicine. As more integral proteins and molecules to this process are discovered, such as neurotrophic growth factors and Cdk2, it is important to note that this is but a small part of the molecular machinery that works in tandem to regulate the remarkably complex process of neuronal formation from neural stem cells. While the full capacity of the neurobiological mechanisms involved with neurogenesis has yet to be fully understood, the possible benefits suggested from developing stem cell-based therapies to rebuild neurons offers an exciting pathway to combat currently incurable neurodegenerative diseases such as Alzheimer’s and Parkinson’s disease. Researchers hope that, with the development of such therapies, damaged or diseased neurons involved in cognitive impairment may be effectively replaced with healthy neurons to ultimately restore normal function in patients. With further research needed, much more remains to be investigated in this field of regenerative medicine before the artificial, neuronal repair of a diseased brain may transition from theory to reality.
Acknowledgements
I’d like to thank my editors Anna Castello and Marley Ottman, of the Berkeley Scientific Journal Features Department, for their thorough edits and guidance throughout the writing process.
References
- Rajabzadeh, N., Fathi, E., & Farahzadi, R. (2019). Stem cell-based regenerative medicine. Stem Cell Investigation, 6, 19. https://doi.org/10.21037/sci.2019.06.04
- Liu, X., Huang, J., Chen, T., Wang, Y., Xin, S., Li, J., Pei, G., & Kang, J. (2008). Yamanaka factors critically regulate the developmental signaling network in mouse embryonic stem cells. Cell Research, 18(12), Art. 12. https://doi.org/10.1038/cr.2008.309
- Cai, C., & Grabel, L. (2007). Directing the differentiation of embryonic stem cells to neural stem cells. Developmental Dynamics, 236(12), 3255–3266. https://doi.org/10.1002/dvdy.21306
- What is neurogenesis? – Queensland Brain Institute—University of Queensland. (n.d.). Retrieved November 7, 2022, from https://qbi.uq.edu.au/brain-basics/brain-physiology/what-neurogenesis
- Kumar, A., Pareek, V., Faiq, M. A., Ghosh, S. K., & Kumari, C. (2019). ADULT NEUROGENESIS IN HUMANS: A Review of Basic Concepts, History, Current Research, and Clinical Implications. Innovations in Clinical Neuroscience, 16(5–6), 30–37.
- Winner, B., & Winkler, J. (2015). Adult Neurogenesis in Neurodegenerative Diseases. Cold Spring Harbor Perspectives in Biology, 7(4), a021287. https://doi.org/10.1101/cshperspect.a021287
- Lacar, B., Young, S., Platel, J.-C., & Bordey, A. (2010). Imaging and Recording Subventricular Zone Progenitor Cells in Live Tissue of Postnatal Mice. Frontiers in Neuroscience, 4. https://www.frontiersin.org/articles/10.3389/fnins.2010.00043
- Kowalczyk, A., Filipkowski, R. K., Rylski, M., Wilczynski, G. M., Konopacki, F. A., Jaworski, J., Ciemerych, M. A., Sicinski, P., & Kaczmarek, L. (2004). The critical role of cyclin D2 in adult neurogenesis. The Journal of Cell Biology, 167(2), 209–213. https://doi.org/10.1083/jcb.200404181
- Ashton, R. S., Conway, A., Pangarkar, C., Bergen, J., Lim, K.-I., Shah, P., Bissell, M., & Schaffer, D. V. (2012). Astrocytes regulate adult hippocampal neurogenesis through ephrin-B signaling. Nature Neuroscience, 15(10), 1399–1406. https://doi.org/10.1038/nn.3212
- Huang, E. J., & Reichardt, L. F. (2003). Trk Receptors: Roles in Neuronal Signal Transduction. Annual Review of Biochemistry, 72(1), 609–642. https://doi.org/10.1146/annurev.biochem.72.121801.161629
- Jurkowski, M. P., Bettio, L., K. Woo, E., Patten, A., Yau, S.-Y., & Gil-Mohapel, J. (2020). Beyond the Hippocampus and the SVZ: Adult Neurogenesis Throughout the Brain. Frontiers in Cellular Neuroscience, 14. https://www.frontiersin.org/articles/10.3389/fncel.2020.576444
- Shohayeb, B., Diab, M., Ahmed, M., & Ng, D. C. H. (2018). Factors that influence adult neurogenesis as potential therapy. Translational Neurodegeneration, 7(1), 4. https://doi.org/10.1186/s40035-018-0109-9
Image References
Figure 3: What is neurogenesis? – Queensland Brain Institute—University of Queensland. (n.d.). Retrieved November 7, 2022, from https://qbi.uq.edu.au/brain-basics/brain-physiology/what-neurogenesis
Figure 4: Editors, B. D. (2017, May 15). Neurogenesis (Adult Psychology)—Definition and Quiz. Biology Dictionary. https://biologydictionary.net/neurogenesis/
Figure 5: Kowalczyk, A., Filipkowski, R. K., Rylski, M., Wilczynski, G. M., Konopacki, F. A., Jaworski, J., Ciemerych, M. A., Sicinski, P., & Kaczmarek, L. (2004). The critical role of cyclin D2 in adult neurogenesis. The Journal of Cell Biology, 167(2), 209–213. https://doi.org/10.1083/jcb.200404181