The Diffraction Limit Dilemma
The chemical messenger of a neuron, a neurotransmitter, is only 0.5-5 nanometers in size. They don’t look like much, but these miniscule molecules are responsible for a cascade of signals that lead to a neuron firing in the brain. Their specific interactions can accumulate into physical motor movements, memory formation, and even drastic changes such as the onset of Parkinson’s disease. Each level of the nervous system, from neurotransmitter to behavioral movement, is connected in an intricate jigsaw. However, visualizing such small structures is no easy feat. This is because most common microscopes that use light are constrained by the diffraction limit, a phenomenon which describes when two objects are close enough to appear as a single blurry spot.
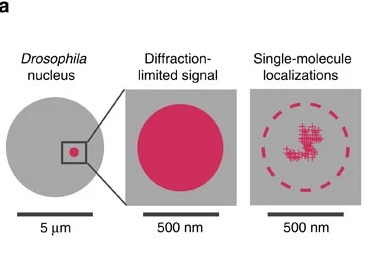
When scientists are capturing an image of a molecule tagged with a fluorescent dye, molecules that are simultaneously emitting light can interfere with each other and provide misleading data about the location and number of molecules. German physicist Ernst Abbe determined that this obstruction occurs when the observed subject is smaller than 500 nm in the z-axis and 200 nm for the x and y- axes.6 These limitations do not hinder visualization of human cells (10,000nm) and even organelle structures within them (1000 nm).7 However, when it comes to examining chromatin and protein structures (10 nm), all that can be visualized is a blurry spot.
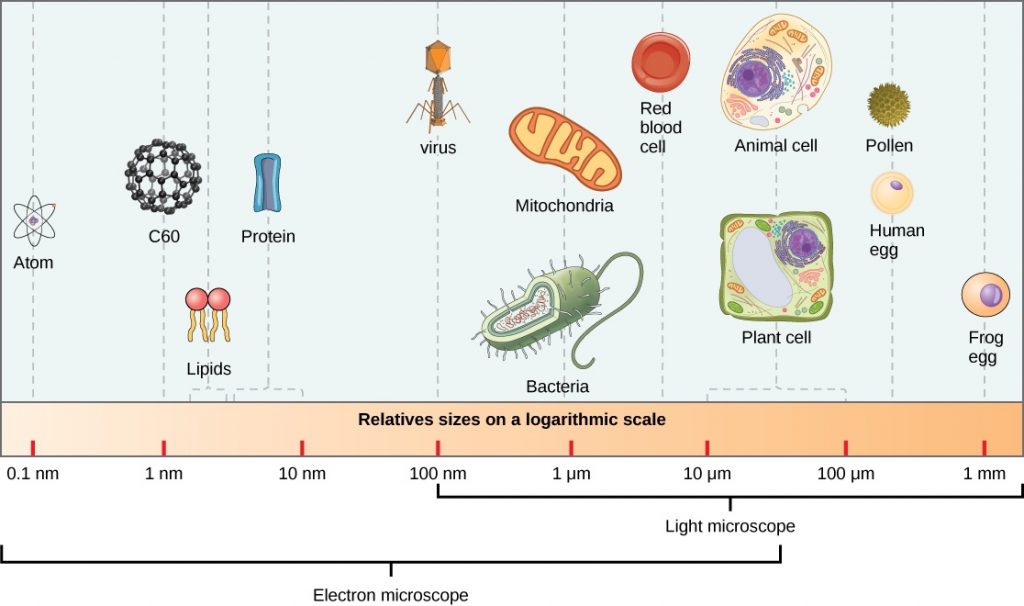
STORM Illuminates Single Molecules
With these limitations in mind, it becomes a question of how we can visualize at the nanometer scale without using light. One popular solution used today is STochastic Optical Reconstruction Microscopy (STORM), which comes from a larger family of super-resolution fluorescence microscopy techniques.4 These are a group of tools used to attach and image fluorescent molecules of interest at a single molecule level. The first discovery of this technique received a Nobel Prize in 2014, and its derivatives, including STORM, have been widely used in the biological field since. The beauty of STORM is that it solves the issues in localizing individual molecules even in dense populations, creating crisp depictions of the structures. Broadly, it does this through stochastically, or randomly, turning on and off fluorescent probes bound to targeted molecules to pinpoint their location. Each probe is built of two main components. One part fuses to the molecule of interest. The second aspect of the probe is a dye that is excited by photons from the wavelengths of visible light (400-700 nm), which are emitted by the microscope. The level of light, or activation intensity, is specifically set so that a subset of the dye molecules will turn on and display fluorescence. As a result, the signals do not substantially overlap at each time point. Then it reconstructs the image in high resolution by combining multiple images from each series of activated probes. Using this sequence of images, small portions of densely populated molecules at a specific point in time can be computationally combined to generate a full image of the molecular landscape.6

Therapeutic Applications of Novel Microscopy
Dyes used in STORM microscopy are capable of binding to proteins, DNA, and RNA, allowing this form of microscopy to be applicable in a wide range of fields. While understanding the structure of something at this small scale can seem insignificant, many discoveries result from conceptualizing the formation and function of these molecules at such high spatial resolutions. In particular, there has been extensive use of this novel technique to study neurological diseases like Parkinson’s.
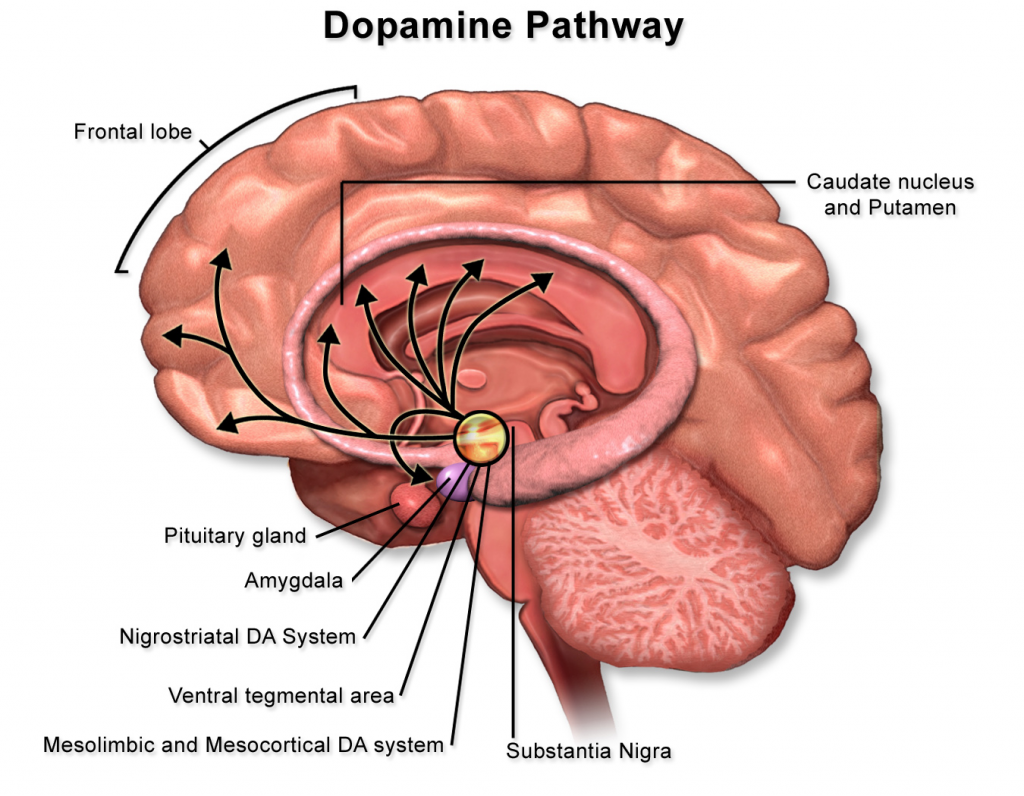
Before STORM, there was still considerable research being done on the brain, including identifying the portions responsible for memories, vision, and motor movement. Scientists over time have comprehensively mapped neuronal networks of the human brain. They have even developed extensive networks linking together the interactions of most genes responsible for neurological diseases, such as Alzheimer’s. However, despite understanding the physical position of neurons and all the genes involved, brain disease treatment is still elusive because of the mystery behind neuronal signaling.
Neurons are connected at the synapse, which is the gap connection through which neurotransmitters pass from one neuron to the next. Interactions between synapses enable neurons to form chain reactions and continue the signal throughout the brain.
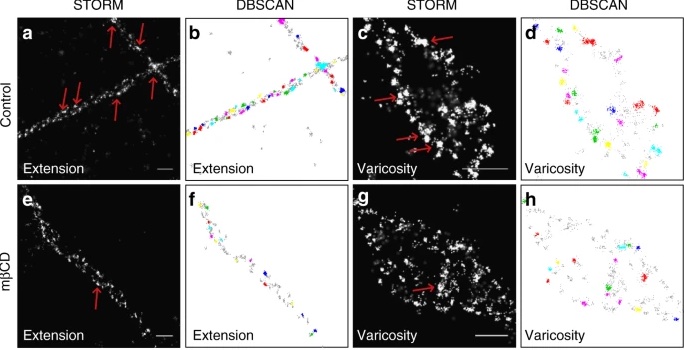
Neurons receive information through receptors, which are protein complexes embedded on the surface of the cell membrane. Maintaining these synapses is incredibly important for neuron health and proper signaling. For example, the dopamine pathway is critical for the brain’s processing of reward, cognitive, and motor function. It is also the primary pathway impacted by Parkinson’s disease. Dopamine is transported through the pathway starting from the substantia nigra, where dopamine is produced, to the striatum of the basal ganglia, a critical structure for bodily movement. Deterioration of the dopamine pathway, as seen in Parkinson’s, can result in tremors, cognitive decline, and movement inhibition. The development of Parkinson’s is in part due to the loss of neurons in the dopamine pathway, especially in the substantia nigra.
At the synapse of dopamine-responsive, or dopaminergic neurons, the dopamine transporter (DAT) plays an important role in dopamine signaling. Its role in the synapse is to capture the released dopamine neurotransmitter and return it to the neuron, preventing the next neuron from being continuously stimulated. Using STORM, these interactions can be visualized and studied at a previously unattainable resolution, revealing synaptic function and transmitter organization at the molecule level. STORM has also revealed that DAT is not evenly distributed across the cell membrane, but is actually confined to nanodomains, or specific protein organizations. Furthermore, scientists have been able to study the interactions of nanometer-scale molecules like cholesterol and excitatory receptor proteins, deducing their role in facilitating the clustering of these nanodomains for DAT. This suggests that these nanodomains can be modulated through excitatory input. There could be further interesting implications regarding the mechanisms of these dopaminergic, or dopamine-responsive, neurons when excited.
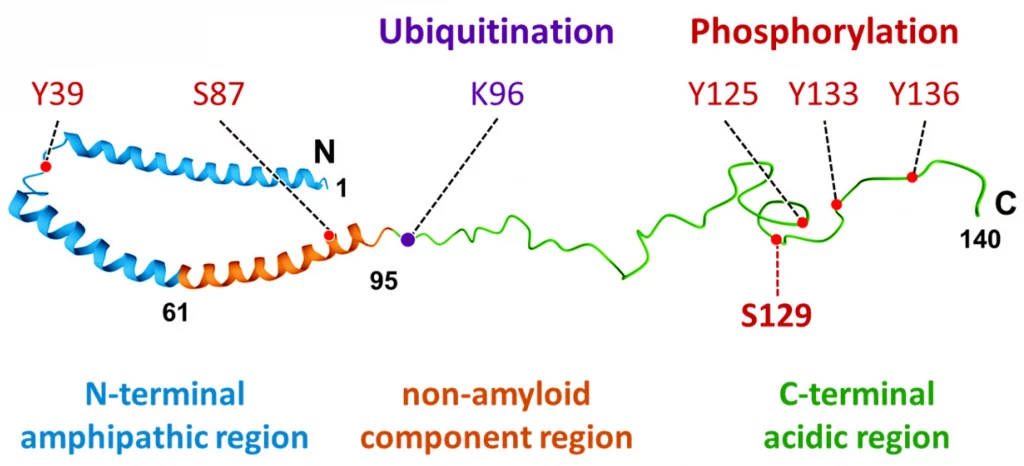
STORM microscopy has been used to receive novel insight into the structures of protein aggregates related to Parkinson’s as well. These proteins have previously been unidentifiable through regular microscopy because the structures are less than 50 nm in size. Using fluorescent probes and STORM, samples of substantia nigra from patients with Parkinson Disease were examined for Lewy bodies, a hallmark of the neurodegenerative disease. Lewy bodies are formed when the molecule α-synuclein becomes phosphorylated (p.α-syn). α-synuclein is typically found in healthy presynaptic terminals involved with neural signaling, but aggregates and becomes toxic to the cell when phosphorylated.3
Lewy bodies contain hundreds of proteins, each only nanometers in size. The structure and formation of these proteins were previously unknown due to the limitations of light-based microscopes. Since STORM is capable of two color imaging with different fluorescent probes, and Lewy bodies are known to be multi-protein, scientists were able to identify the internal molecular structure of these aggregates at high resolution. They found that p.α-syn is bound by neurofilaments and dense honeycomb structures of Lewy bodies.1 Understanding these structures could potentially reveal the underlying mechanisms for their organization and role in the development of neurodegenerative diseases, including Parkinson’s.
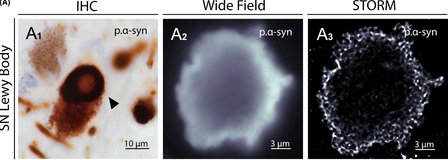
STORM microscopy makes it possible to study Parkinson’s disease from multiple magnitudes. The uncovered interplay of these different protein interactions can then be pieced together to develop the entire mechanism of this neurodegenerative disease. With the power of STORM and other super-resolution fluorescence microscopy techniques, researchers are able to visually understand biology at a molecular level. While Parkinson’s Disease is just one avenue of research in which the technique has been applied, this form of microscopy has untold potential across various biological fields.
Acknowledgements
I would like to thank UC Berkeley public health professor Dr. William Jagust and the BSJ editorial staff for generously reviewing my article for accuracy.
References
- Codron, P., Letournel, F., Marty, S., Renaud, L., Bodin, A., Duchesne, M., Verny, C., Lenaers, G., Duyckaerts, C., Julien, J. P., Cassereau, J., & Chevrollier, A. (2021). STochastic Optical Reconstruction Microscopy (STORM) reveals the nanoscale organization of pathological aggregates in human brain. Neuropathology and applied neurobiology, 47(1), 127–142. https://doi.org/10.1111/nan.12646
- Dani, A., Huang, B., Bergan, J., Dulac, C., & Zhuang, X. (2010). Superresolution imaging of chemical synapses in the brain. Neuron, 68(5), 843–856. https://doi.org/10.1016/j.neuron.2010.11.021
- Kawahata, I., Finkelstein, D. I., & Fukunaga, K. (2022). Pathogenic Impact of α-Synuclein Phosphorylation and Its Kinases in α-Synucleinopathies. International journal of molecular sciences, 23(11), 6216. https://doi.org/10.3390/ijms23116216
- Lelek, M., Gyparaki, M.T., Beliu, G. et al. Single-molecule localization microscopy. Nat Rev Methods Primers 1, 39 (2021). https://doi.org/10.1038/s43586-021-00038-x
- Rahbek-Clemmensen, T., Lycas, M.D., Erlendsson, S. et al. Super-resolution microscopy reveals functional organization of dopamine transporters into cholesterol and neuronal activity-dependent nanodomains. Nat Commun 8, 740 (2017). https://doi.org/10.1038/s41467-017-00790-3
- Rust, M. J., Bates, M., & Zhuang, X. (2006). Sub-diffraction-limit imaging by stochastic optical reconstruction microscopy (STORM). Nature methods, 3(10), 793–795. https://doi.org/10.1038/nmeth929
- “The Relative Scale of Biological Molecules and Structures.” Nature News, Nature Publishing Group,www.nature.com/scitable/content/the-relative-scale-of-biological-molecules-and-14704956/. Accessed 6 Oct. 2023.
Image References
Banner Image: Cultured Rat Hippocampal Neuron. (n.d.). Retrieved October 9, 2023, from https://openverse.org/image/37e92992-4cbd-41c6-8cce-061e90ea65c4
Figure 1: Super-resolution imaging with Oligopaints and STORM. | Nature Communications. (n.d.). Retrieved October 9, 2023, from https://www.nature.com/articles/ncomms8147/figures/2
Figure 2: File:Relative sizes of microscopic entities 2.jpg—Wikipedia. (2013, March 23). https://commons.wikimedia.org/wiki/File:Relative_sizes_of_microscopic_entities_2.jpg
Figure 3: 3D_Dual_Color_Super_Resolution_Microscopy_Cremer_2010.png (3486×1280). (n.d.). Retrieved October 9, 2023, from https://upload.wikimedia.org/wikipedia/commons/1/1a/3D_Dual_Color_Super_Resolution_Microscopy_Cremer_2010.png
Figure 4: Bruce Blaus. (2017). English: An illustration showing the dopamine pathway. Own work. https://commons.wikimedia.org/wiki/File:Dopamine_Pathway.png
Figure 5: Rahbek-Clemmensen, T., Lycas, M.D., Erlendsson, S. et al. Super-resolution microscopy reveals functional organization of dopamine transporters into cholesterol and neuronal activity-dependent nanodomains. Nat Commun 8, 740 (2017). https://doi.org/10.1038/s41467-017-00790-3
Figure 6: Kawahata, I., Finkelstein, D. I., & Fukunaga, K. (2022). Pathogenic Impact of α-Synuclein Phosphorylation and Its Kinases in α-Synucleinopathies. International journal of molecular sciences, 23(11), 6216. https://doi.org/10.3390/ijms23116216
Figure 7: Codron, P., Letournel, F., Marty, S., Renaud, L., Bodin, A., Duchesne, M., Verny, C., Lenaers, G., Duyckaerts, C., Julien, J. P., Cassereau, J., & Chevrollier, A. (2021). STochastic Optical Reconstruction Microscopy (STORM) reveals the nanoscale organization of pathological aggregates in human brain. Neuropathology and applied neurobiology, 47(1), 127–142. https://doi.org/10.1111/nan.12646