At the heart of what Einstein’s theory of general relativity tells us about the Universe is a question posed over a hundred years ago: what lies inside the event horizon of a black hole, and how can we mathematically describe it? How do we study the evolution of a dark object that swallows light and matter? The field of black hole physics is still rife with mystery; however, gravitational wave experiments have provided a groundbreaking lens of observation.
The Fundamentals
Einstein’s theory of general relativity, formulated in 1916, relies on fundamental concepts from special relativity, where three-dimensional space and one-dimensional time are not separate metrics, but rather comprise one four-dimensional cosmic fabric—spacetime. Because we view the world in the three-dimensional x-y-z plane, it is impossible for our minds to physically picture a four-dimensional space. We can instead visualize spacetime as a giant cloth, stretched above the ground, holding objects of varying mass.
As seen in Figure 1, a sphere of greater mass will create a greater disturbance in the cloth compared to a sphere of smaller mass. This curvature caused by high mass objects also alters the path of incoming objects, causing them to fall into orbit around a high mass object rather than pass by in a straight line. This effect is analogous to gravity. Instead of describing gravity as a force pulling objects toward one another in the cosmos, general relativity theorizes that it is an acceleration due to the curvature or warp in spacetime dictated by the presence of mass and energy. This curved spacetime then informs the motion of other objects. For example, the path of light, wave-like particles called photons, will bend with the curvature of spacetime around extremely massive objects on its way to the observer.1 In this sense, the gravitational force is embedded in the geometry of the Universe.
What are black holes?
At the end of some massive stars’ lives, a cosmic pit emerges from which inside a certain radius called the “event horizon,” anything with a finite speed, such as matter and even light itself, cannot escape—hence the term “black hole.” These objects begin as massive stars powered by burning hydrogen into helium in a process called nuclear fusion. Nuclear fusion occurs in the core of the star, creating an outward pressure that counters the star’s self-generated gravity. While the star lives, these two forces balance against each other in equilibrium. Fusion continues to create heavy elements in the cores of massive stars until the star has built up an iron core.
When the star has fused the last of its “fuel,” the outward pressure from nuclear fusion no longer acts against the gravity of the star, beginning a phase of rapid collapse. The falling layers of the star rebound on the contracting core, producing an extreme shockwave through the material, signaling the start of what is called a supernova explosion. While the outer layers continue blasting outward, scattering elements into the interstellar medium, the core of the massive star continues to collapse inward, compressing its matter into a smaller and smaller volume of space until a gravitational singularity is formed—a point in spacetime where density is infinite. If we were to place the black hole singularity into our initial fabric analogy, the object would create an infinite well in the cloth (Figure 2) as predicted by general relativity, and analogously, an infinite curvature in spacetime.
Because black holes cannot be “seen” in the same sense as a luminous star (Figure 3), scientists must probe the properties–masses and spins (angular momentum)–of these objects using different methods. Results obtained using gravitational wave observations of binary black holes have yielded extraordinary results.
Let’s go back to the cloth model. Einstein’s theory of general relativity additionally predicts a unique phenomenon in the case of high energy events. One example is a system of massive binary objects like black holes and neutron stars orbiting and accelerating toward each other.2 The rotation of these gravitationally bound objects creates ripples in the fabric of spacetime that propagate at the speed of light, creating stronger and stronger undulations as the objects near collision.3 These are gravitational waves (Figure 4).
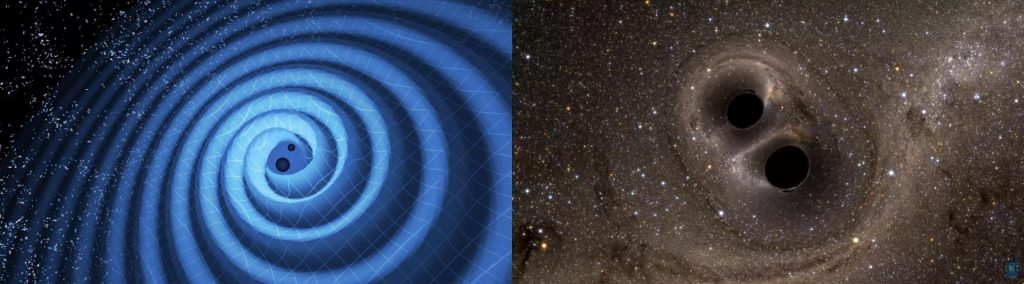
What if we could study black holes by this gravitational signal? On September 14, 2015, this avenue of exploration became possible. After decades of development and almost a century after Albert Einstein theorized gravitational waves, the Laser Interferometer Gravitational-Wave Observatory (LIGO) detected a signal produced moments before two spinning black holes merged with one another approximately 1.3 billion light years (410-180+160 Mpc) away.4 This extraordinary detection made during LIGO’s engineering run earned Barry Barish, Rainer Weiss, and Kip Thorne the 2017 Nobel Prize in Physics. A numerical simulation of the merger event (Figure 4) depicts a lensing effect around the black holes caused by their extreme gravity. The massive objects severely warp spacetime, altering the path of light from surrounding objects like dust and other stars.
LIGO consists of two detectors (Figure 5) in Hanford, Washington, and Livingston, Louisiana. These 4 km-long interferometers are sensitive to the fluctuations in spacetime produced by gravitational waves through vibrations that alter the length of each reflected laser arm by approximately 1/200 of the radius of a proton.5
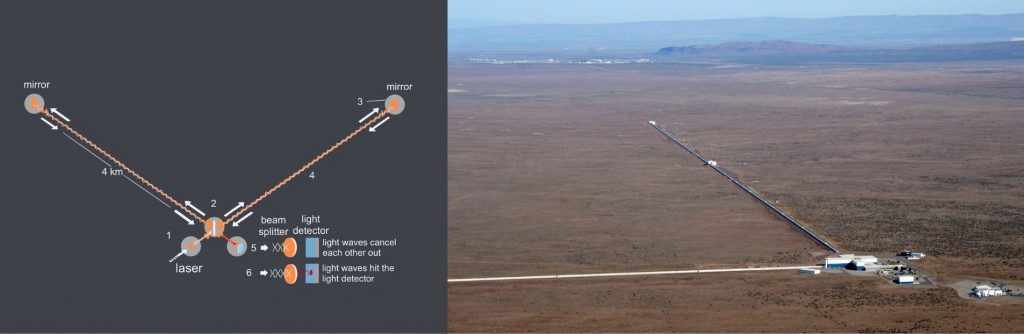
The captured signal, GW150914, was first “heard” by Hanford (H1), then Livingston (L1) with a 6.9-0.4+0.5 ms delay due to the time the signal takes to travel from H1 to L1. The strain, depicted in Figure 6, which is the fractional change in the LIGO arm lengths, increases over time, maximizing in amplitude before the coalescence between the two black holes. It then sharply decays at the “ring-down,” the signal produced by the final black hole formed in the merger.4, 5 Also depicted are the dual signals as frequencies, sharply increasing at the point of collision. From the gravitational wave signature, scientists are able to infer properties about the two binary counterparts such as black hole mass and spin (angular momentum). For the historic GW150914 black holes, mass constraints of 36-4+5M and 29-4+4M were calculated with a final black hole mass of 62-4+4M. The remaining 3M was radiated away in gravitational wave energy (M= the mass of the Sun). The detection of these binary black holes by gravitational waves was a major achievement that not only supports Einstein’s theories, but also filled in gaps in our understanding of black hole physical properties, doubling the known mass range of black holes and confirming the existence of black holes heavier than 25 M.4, 5
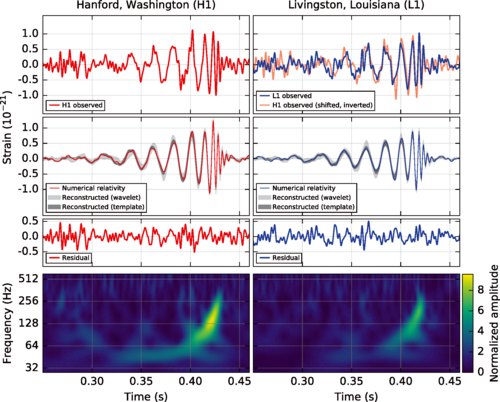
LIGO has since completed three observing runs, with the Advanced Virgo interferometer in Cascina, Italy, joining the fray in August 2017. Around 70 black hole mergers have been detected over the course of these runs, the last of which ended in March 2020.6 Physicists are particularly interested in the masses and spins of the black holes because these properties are crucial to studying how these objects were formed in the first place. For example, distinctions in the masses and spins as deduced by the gravitational wave signal may indicate that the system originated from one of two evolutionary processes: isolated binary evolution or dynamical formation.6 The first describes merger counterparts that evolved from two supernova deaths of massive stars into black holes (or neutron stars), and the latter describes counterparts that fell into orbit with one another in exceedingly dense regions of galaxies, such as star clusters.
With each observing run, scientists can probe the properties of black holes with growing precision. However, elements of black hole evolution remain shrouded in mystery and require more data to further explore. A couple examples are theorized lower and upper mass gaps in the range of detected binary systems.6 These gaps would describe the astrophysical processes of how massive stars die: whether they form neutron stars, black holes, or perhaps no remnant at all. Great potential lies ahead to breach these gaps in our knowledge with LIGO’s fourth observing run (O4), which officially commenced in May 2023, united with the Virgo and KAGRA interferometers in Italy and Japan.7 O4 will run for 18 months, equipped with recent instrumental upgrades to increase detector sensitivity to distant gravitational wave sources.
The story of black hole evolution has spanned over a century, with gravity and instrumentation at the focal point. It is an incomplete puzzle, coming together piece by piece the farther we look back in time at distant black holes deep in the Universe.
Acknowledgements
Thank you to Dr. Raffaella Margutti, Associate Professor of Astronomy and Physics at UC Berkeley, for first inspiring me to explore multimessenger astrophysics and for reviewing this article.
Figures
References
[1] Einstein, A. (1936). Lens-like action of a star by the deviation of light in the gravitational field. Science, 84(2188), 506-507.
[2] Einstein, A. Näherungsweise Integration der Feldgleichungen der Gravitation. Sitzungsber. K. Preuß. Akad. der Wiss. 1916, 688–696 (1916).
[3] Abbott, B. P., Abbott, R., Abbott, T. D., Acernese, F., Ackley, K., Adams, C., … & Callister, T. A. (2017). Gravitational waves and gamma-rays from a binary neutron star merger: GW170817 and GRB 170817A. The Astrophysical Journal Letters, 848(2), L13.
[4] Abbott, B. P., Abbott, R., Abbott, T. D., Abernathy, M. R., Acernese, F., Ackley, K., … & Cavalieri, R. (2016). Observation of gravitational waves from a binary black hole merger. Physical review letters, 116(6), 061102.
[5] Miller, M. C., & Yunes, N. (2019). The new frontier of gravitational waves. Nature, 568(7753), 469-476.
[6] Abbott, R., Abbott, T. D., Acernese, F., Ackley, K., Adams, C., Adhikari, N., … & Boudart, V. (2021). The population of merging compact binaries inferred using gravitational waves through GWTC-3. arXiv preprint arXiv:2111.03634.
[7] Latest update on start of Next observing run (o4). Caltech. (2023, January 21). Retrieved April 5, 2023, from https://www.ligo.caltech.edu/LA/news/ligo20220123#:~:text=After%20over%20two%20years%20of,will%20begin%20in%20May%202023.
Image References
Figure 1: Adapted from ESA – C.Carreau (2015, January 5) https://www.esa.int/ESA_Multimedia/Images/2015/09/Spacetime_curvature
Figure 2: Phoebe Mak (May 18th, 2023)
Figure 3: Event Horizon Telescope Collaboration. (2019). First M87 event horizon telescope results. I. The shadow of the supermassive black hole. arXiv preprint arXiv:1906.11238.
Figure 4: (left) LIGO, T. Pyle https://www.space.com/gravitational-waves-memory-space-time.html (right) Simulating Extreme Spacetimes Project https://www.black-holes.org/
Figure 5: (left) Phoebe Mak (May 18th, 2023); (right) Caltech/MIT/LIGO Lab (May 2nd, 2008) https://www.ligo.caltech.edu/page/observatories-collaborations
Figure 6: Abbott, B. P., Abbott, R., Abbott, T. D., Abernathy, M. R., Acernese, F., Ackley, K., … & Cavalieri, R. (2016). Observation of gravitational waves from a binary black hole merger. Physical review letters, 116(6), 061102.